

There is currently a great deal of commercial interest in the use of EB curing for packaging and industrial applications. For adhesive applications, the technology offers the potential benefits of immediate cure, reduced work-in-progress, reduced energy consumption, reduced floor space, no solvent use and no isocyanates1,2. Additionally, EB-curable coatings have been used commercially as topcoats for 20 years, and interest continues to grow. In addition to the aforementioned benefits, EB-curable coatings also reportedly yield increased gloss, greater process robustness and the potential for faster line speeds vs. other coatings technologies.
EB-curable laminating adhesives and coatings are now available commercially to function on a variety of substrate combinations. The development of EB-curable adhesives to satisfy the demanding conditions of many well-entrenched applications is not a trivial exercise however. Adhesion scientists must address many hurdles to realize the potential benefits of the technology.
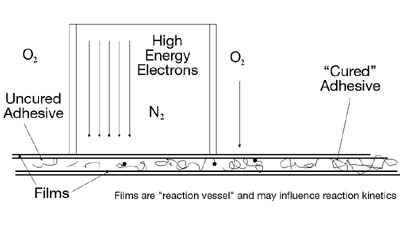
Elements of Adhesion
Adhesion is a complex phenomenon requiring the interrelated steps of- 1. Wetting (related to surface energetics),
2. Macromolecular flow and deformation during bonding,
3. Specific chemical interactions, and
4. Resistance to deformation and energy dissipation during debonding.
The role of surface energetics in adhesion is well documented3,4. Although a great deal of work has been done detailing the role of viscoelasticity in adhesion for pressure sensitive adhesives (PSAs)5-7, relatively little work has been reported for laminating adhesives and virtually none for energy-curable laminating adhesives.
For EB-curable adhesives, seemingly subtle changes in process conditions (e.g., EB dose and line speed) and/or formulation (e.g., Tg of monomer, level of multifunctional monomers) may produce rather dramatic responses in extent of cure and therefore changes in the viscoelastic character of the adhesive. Substrate interactions may influence the cure rate as well. These changes may all influence adhesion. A detailed review of the role of viscoelasticity in EB-cured adhesives is therefore helpful in understanding the adhesion process.
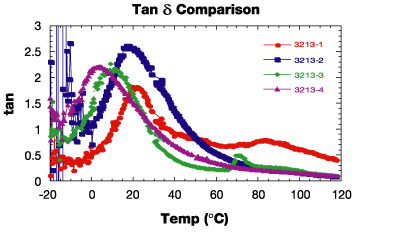
Surface Energetics in Adhesion
The role of surface energetics in adhesion can be conveyed with the analogy of water beading up on a freshly waxed car vs. spreading out on an unwaxed car. When freshly waxed, the surface energy of the car has been greatly reduced by the application of hydrocarbon wax. In such a case, the water (high-surface-tension liquid) cannot wet out the surface since the water molecules have a greater attraction for one another than for the surface.
This lack of wetting is good for water on a car surface (reduced corrosion, dirt pick-up), but the analogous situation for an adhesive would be undesirable (reduced adhesion). To overcome the unfavorable energetics, the adhesive scientist could increase the surface energy of the film (corona treatment or chemical treatment) or adjust the formulation to yield a lower surface energy. Krawiec8 gives a practical discussion of the role of surface energetics in adhesion for PSAs.
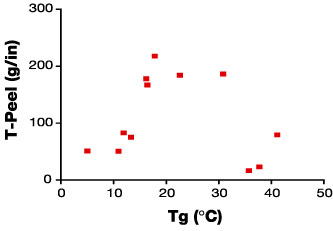
Specific Chemical Interactions in Adhesion
Once favorable surface energetics have been achieved (wetting), adhesion may be further enhanced through specific chemical interactions between the adhesive and the substrate surface. Adhesion-promoting additives are used to enhance this specific or chemical adhesion. These interactions may be covalent bonds, hydrogen bonds or dipole-dipole interactions.
Covalent bonds between adhesive and substrate are the strongest of the interactions, but appreciable adhesion enhancement is also achievable through hydrogen bonds and even dipole-dipole interactions. Fowkes9 showed that adhesion of polymethyl methacrylate and chlorinated polyvinyl chloride to glass is dramatically affected by whether the glass is acidic or basic. In doing so, what was previously assumed to be a dipole-dipole interaction was instead shown to be a Lewis acid-base interaction (not attributable to dipole moment but the interaction of acid and basic sites).
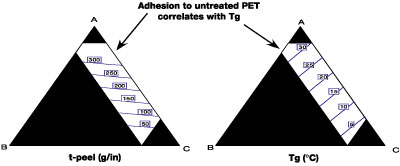
Viscoelasticity in Pressure Sensitive Adhesives
A great deal of pioneering work in the field of viscoelasticity and adhesion has been done for PSAs. Dahlquist10 stated a PSA must by definition satisfy the rheological requirement that the 1-sec creep compliance (J) be greater than 1 x 10-6 cm2/dyne at use temperature. This definition translates into a storage modulus (G') of less than 1x107 dyne/cm2. More recently, Chu and Class11 published rheological "windows" in an attempt to define specific targets for Tg and G' at 25 deg C for PSAs for various applications. (See Figure 1.)Such attempts recognize that for a pressure sensitive adhesive, macromolecular flow and deformation (requiring a low modulus) must take place during application of the adhesive (solid)-coated substrate to a second surface. This flow and deformation is a result of molecular segmental motion. Techniques such as Dynamic Mechanical Analysis (DMA) can be used to document the relatively low modulus (G') at ambient conditions for PSAs.
Additionally recognized by this approach is the fact that the PSA must exhibit sufficient resistance during debonding or stress. This resistance to debonding requires that the PSA exhibit stiffness or a high modulus under the debonding conditions. Since debonding is a relatively high-rate process and bonding is a relatively low-rate process, a PSA that is "properly tuned" from a viscoelastic standpoint appears soft when bonding but harder when debonding due to the time-temperature superposition principle. The storage modulus increases by several orders of magnitude at the Tg; hence, the relevance of the Tg to the stiffness requirement.

EB-Curable Laminating
Electron beam-curable laminating adhesives are applied as liquids or "syrups" onto a moving primary film web at very low application weights (1 lb to 2 lb/ream). A second film web is then immediately brought into contact with the primary web, and the three-layer structure is subsequently passed into the nitrogen-flooded EB chamber. (See Figure 2.) Inside the chamber, high-energy electrons pass through the construction to a depth governed by the applied voltage. To pass through the films used in laminating (0.5 mil to 1 mil), as well as the adhesive (0.1 mil), typically a voltage of at least 100 kV is needed. The high-energy electrons initiate polymerization of the reactive components within the adhesive. Upon exiting the chamber, the formerly liquid adhesive is now a viscoelastic solid.
Viscoelasticity in Laminating Adhesives
Conventional PSAs are applied as solid films (i.e., adhesive film on tapes and labels) to a second substrate (e.g., container) with pressure and therefore must exhibit macromolecular flow and deformation in their solid or polymerized state to give tack and adhere to substrate. On the other hand, EB-curable laminating adhesives must exhibit macromolecular flow and deformation in their pre-polymerized state (i.e., in their liquid state when applied to the moving web).Restated, the pre-polymerized laminating adhesive must flow out evenly over the film when applied by the application method in use (gravure, flexo, reverse roll, etc.) at the required line speeds. Measurements of low-shear viscosity give a rough idea of prepolymer flow, but more complex rheological determinations give a better picture. If the surface energetics are favorable and the rheology is optimized, a uniform adhesive film of desirable coat weight can be achieved.
Once polymerized, however, laminating adhesives need to exhibit a viscoelastic response not unlike that of PSAs. Specifically, they must offer resistance at fast rates of deformation and exhibit sufficient energy dissipation to help avoid stress concentration at the adhesive-substrate interface. For many applications, laminating adhesives must additionally offer exceptional resistance at low rates of deformation at both ambient and elevated temperatures. They must do so while functioning at very low application weights. Therefore, it is not unexpected that the viscoelastic requirements for EB-curable laminating adhesives bear some vague similarity to that of PSAs while being clearly differentiated due to the different application process and different performance requirements.
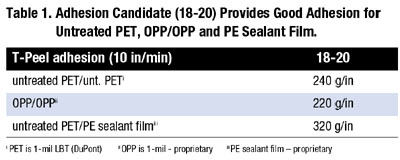
EB-Curable Laminating-Adhesive Development
If one were to naively attempt to utilize a radiation-curable coating formulation as a laminating adhesive, the result would most likely be poor adhesion. This is a result of the fact that although coatings need adhesion to one surface, they also need to have abrasion resistance, impact resistance and a variety of properties unique to the specific application.The need for abrasion resistance largely dictates that coatings have a Tg above ambient conditions and a sufficiently high modulus (stiffness) at room temperature to avoid becoming soft or deformable in use. The high Tg is achieved through use of monomers with the appropriate Tg. The stiffness is a manifestation of the higher Tg and a fairly high crosslink density.
The high Tg and stiffness (high storage modulus) of coatings in most cases results in a low elongation and poor energy dissipation when stressed at ambient temperatures. As a result, if the coating formulation were bonded between films (and possessed appropriate surface energetics and flow to provide an intimate initial bond) and the films were subsequently pulled apart, most of the applied energy would be absorbed not by the adhesive but instead transferred to the bond line. The result would be low bond strength (zipping) unless the adhesive had remarkable specific chemical interaction with the substrate.
Laminating adhesives by comparison are typically characterized by a lower Tg and a lower modulus at ambient temperatures than coatings to allow for more effective energy dissipation. The Tg is ideally near the use temperature since energy dissipation is maximized at the Tg (maximum of ratio of loss modulus G" to storage modulus G'). Energy dissipation is also maximized by a lower crosslink density.
EB curing complicates the picture, however. The curing of an EB-curable adhesive between films is a free-radical polymerization process. As the polymerization proceeds, molecular weight builds and crosslinking ensues. The modulus and Tg are therefore changing throughout the curing process. The extent of final cure has an impact on viscoelasticity and, hence, adhesion.
Although it is obvious that under-cured adhesives are undesirable for extraction, odor and aesthetics, under-curing also impacts adhesion and heat resistance. Incomplete cure results in a reduction of Tg and tensile properties due to the plasticizing effect of unreacted monomer. It also results in lower molecular weight and a lower crosslink density, which reduce modulus.
Conversely, over-cure also has detrimental effects on adhesion. In addition to the potential to change the performance of some films12, application of too high a dose can result in a more highly crosslinked network within the adhesive than is desired. The tight network reduces the ability to dissipate energy and yield; hence, stress is concentrated at the bond line. Interestingly, it is surmised that grafting reactions may also be initiated to some substrates by over-cure, which may improve adhesion through specific chemical interaction.
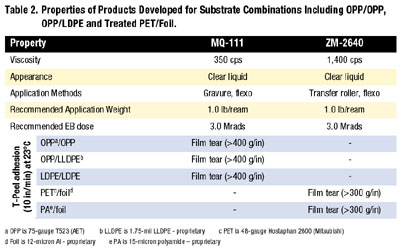
Effect of Tg and Modulus Upon Adhesion
The relationship of the Tg of the adhesive to T-peel adhesion for EB-curable laminating adhesives can be clearly demonstrated. Specifically, this has been done by the preparation, application between films and curing of a series of formulations differing in Tg. The adhesives were characterized rheologically using a Rheometrics Dynamic Analyzer RDA-2 (Figure 3) and tested for adhesion.Specifically, the adhesion testing was carried out by applying the formulations online onto untreated PET and married to a second film of the same, and then cured at 3 Mrad @ 20 ft/min. The constructions were then tested for T-Peel adhesion at 10 in/min crosshead speed. As can be seen in Figure 4, there is clearly a Tg threshold for adhesion to untreated 92-gauge PET, beyond which adhesion drops off dramatically.
The time-temperature superposition principle suggests that higher rates of testing will lower the optimal Tg of the adhesive. It should be emphasized that the choice of film (type, treatment level and thickness) is also expected to impact the optimal viscoelasticity for adhesion. Some films are capable of a higher level of energy dissipation. For such films, the viscoelasticity of the adhesive may be less strongly correlated with adhesion than for PET.
In addition to the aforementioned dependency of adhesion upon Tg, there is also an analogous optimal region for storage modulus (G'). In general, laminating adhesives are characterized as having higher G' at 25 deg C than that for PSAs. With too high a modulus (high crosslink density), energy dissipation is reduced. When the modulus is too low, strength and heat resistance are inadequate.
Robust Performance
Advanced EB-curing units deliver a fairly uniform dose across the web. They also compensate for line-speed changes by adjusting the delivered dose to the substrates. Nevertheless, the extremes of production encountered in high-speed film laminating as well as the variety of films encountered can be expected to induce subtle changes in the extent of cure of the adhesive. Commercially viable adhesives must be robust enough to handle these extremes.A more fundamental, inherent challenge to uniformity exists for EB curing, however. Unlike conventional free-radical polymerizations in which radicals are slowly generated over time, EB curing generates a tremendous number of radicals at one instant. The mechanism for the generation of free radicals by EB is well documented13. As line speeds increase and dose is held constant, these radicals are generated over a shorter and shorter span of time.
Changing the rate of radical generation can have profound effects on free-radical polymerization kinetics. The propagation rate of free-radical polymerization is directly proportional to the concentration of free radicals, while chain termination is proportional to the free-radical concentration squared14. Higher free-radical concentration favors chain termination, which in turn can affect conversion and final polymer architecture. Substrates may also increase or decrease cure rate by either directly or indirectly participating in the reaction or quenching generated radicals. A commercially viable adhesive must be robust enough to deliver the same adhesion as conditions change.
EB-Curable Adhesive Optimization
The challenge in developing a viable EB-curable adhesive for laminating is therefore to tailor a system that:- 1. Exhibits the correct wetting (surface energetics) for the substrates used,
2. Exhibits the correct flow (viscosity) on the application equipment used,
3. Maximizes the chemical interactions (specific adhesion),
4. Cures to a desired Tg and modulus for energy dissipation (viscoelasticity), and
5. Functions under a variety of conditions and on a variety of substrates (robust).
Design of experiment (DOE) techniques provide an efficient method for constructing formulation experiments and for identifying the most desirable formulation. DOE can furthermore be coupled with knowledge of the viscoelastic requirements for laminating and useful adhesion-promotion techniques to enhance the product-development process. In the following example (Figure 5), an experimental design was carried out to determine the best formulation for adhesion to untreated PET. In this design, the type and concentration of oligomer was held constant and the amount of three other components (A, B and C) was varied. The use of untreated PET (92-gauge LBT - DuPont) presents a surprisingly demanding challenge for EB laminating adhesives.
The design shows that excellent adhesion (325 g/in) is achieved as the percentage of the higher Tg component A is increased. A strong correlation of adhesion with Tg is noted. As an outgrowth of this design space, a robust adhesion candidate (18-20) was identified with good adhesion for untreated PET, OPP/OPP and PE sealant film (Table 1).
Optimizing an adhesive based on the viscoelastic character of the adhesive alone is, however, many times insufficient to yield a commercially viable product. This is shown by data generated for a series of EB adhesive candidates for an application that requires both ambient and elevated temperature (65 deg C) adhesion to a proprietary substrate combination, as well as low viscosity, fast cure speed and low odor upon cure. As shown in Figure 6a, optimal adhesion at 23 deg C (room temperature) is achieved with formulations exhibiting Tg near 20 deg C. Figure 6b, however, shows such formulations have poor adhesion at 65 deg C. To maximize adhesion at 65 deg C, a Tg of 50 deg C to 60 deg C appears optimal.
To overcome this apparent limitation of the viscoelastic approach, product developers must rely on other adhesion-promotion techniques such as enhanced hydrogen bonding and chemical grafting. When these approaches are pursued simultaneously with viscoelastic optimization, robust adhesion performance on a variety of substrates across a wide temperature range can be achieved.
Using a combination of viscoelastic optimization and other adhesion-promotion techniques, products have been developed for other substrate combinations including OPP/OPP, OPP/LDPE and treated PET/foil. The typical properties of these products are shown in Table 2. These products exhibit a broad adhesion profile to several substrates as well as other necessary performance requirements such as elevated temperature adhesion.
A Holistic Approach
In conclusion, development of EB-curable laminating adhesives requires a holistic approach. In optimizing the performance of the final cured adhesive, consideration must be given to not only selecting the appropriate monomers for film wetting, low odor, desirable toxicology and formulation compatibility but also the resultant viscoelasticity of the adhesive upon cure. A good understanding of the impact of process and substrate changes upon extent of cure and therefore viscoelasticity is also critical to allow for control of adhesion and the design of robust adhesives. This viscoelastic information must, however, be coupled with other useful adhesion-promotion techniques to produce a commercially viable adhesive.Acknowledgments
The authors wish to thank Dr. San Lee, Dr. Alan Nakatani and Mark Swain for assistance offered on this project.
This article is based on a presentation made at RADTECH 2002, Indianapolis. Copyright c 2002, RadTech International N.A., 6935 Wisconsin Ave, Suite 207, Chevy Chase, MD 20185. Reprinted with permission from the RadTech 2002 Conference Proceedings.
References
1. Mykytiuk, A. Flexible Packaging, February 2000, p. 20-23.
2. Mytkytiuk, A. Flexible Packaging, August 2000, p. 16-19.
3. Patton, T.C. TAPPI (1970), 53 (3), 421-9.
4. Zisman, W.A. J. Paint Technol. (1972), 44(564), 41-57.
5. Class, J.B. and Chu, S.G. "The Viscoelastic Properties of Rubber-Resin Blends", J. of Applied Poly. Sci., 30, 805-14; 815-24; 825-42, February 1985.
6. Chu, S.G. Handbook of Pressure Sensitive Adhesive Technology, D. Satas, Ed., Chapter 8, Van Nostrand Reinhold Co., New York (1989).
7. Zosel, A. Colloid Polym. Sci. 263, 541-553 (1985).
8. Kraweic, R. Handbook of Pressure Sensitive Adhesive Technology, D. Satas, Ed., Chapter 22, Van Nostrand Reinhold Co., New York (1989).
9. Fowkes, F.M. et al. J. Polym. Sci. Polym. Chem. Ed. 22, 547-566 (1984).
10. Dahlquist, C.A. Proc. Nottingham Conf. On Adhesion: Fundamentals and Practice. MacLaren, London, 1966.
11. Chu, S.G. Handbook of Pressure Sensitive Adhesive Technology, D. Satas, Ed., Chapter 8, Van Nostrand Reinhold Co., New York (1989).
12. Rangwalla, I. and Maguire, E. RadTech Report, May/June 2000.
13. Odian, G. Principles of Polymerization, 2nd edition, John Wiley and Sons, New York, 1981 p. 212.
14. Ibid. p.189